Torque is a force that makes an object rotate. A nut turns because of the torque applied by a spanner. The si
ze of the torque is found by multiplying the applied force by its distance from the axis, so that a longer spanner exerts greater torque.
Torque, moment or moment of force (see the terminology below), is the tendency of a forceto rotate an object about an axis, fulcrum, or pivot. Just as a force is a push or a pull, a torque can be thought of as a twist.
Loosely speaking, torque is a measure of the turning force on an object such as a bolt or aflywheel. For example, pushing or pulling the handle of a wrench connected to a nut or bolt produces a torque (turning force) that loosens or tightens the nut or bolt.
The symbol for torque is typically τ, the Greek letter tau. When it is called moment, it is commonly denoted M.
The magnitude of torque depends on three quantities: the force applied, the length of the lever arm connecting the axis to the point of force application, and the angle between the force vector and the lever arm. In symbols:


where
- τ is the torque vector and τ is the magnitude of the torque,
- r is the displacement vector (a vector from the point from which torque is measured to the point where force is applied), and r is the length (or magnitude) of the lever arm vector,
- F is the force vector, and F is the magnitude of the force,
- × denotes the cross product,
- θ is the angle between the force vector and the lever arm vector.
The length of the lever arm is particularly important; choosing this length appropriately lies behind the operation of levers, pulleys, gears, and most other simple machines involving a mechanical advantage.
The SI unit for torque is the newton meter (N·m).
Definition and Relation to Angular Momentum
Torque is defined about a point not specifically about axis as mentioned in several books.
A force applied at a right angle to a lever multiplied by its distance from the lever's fulcrum (the length of the lever arm) is its torque. A force of three newtons applied two metres from the fulcrum, for example, exerts the same torque as a force of one newton applied six metres from the fulcrum. The direction of the torque can be determined by using the right hand grip rule: if the fingers of the right hand curl in the direction of rotation and the thumb points along the axis of rotation, then the thumb also points in the direction of the torque.
More generally, the torque on a particle (which has the position r in some reference frame) can be defined as the cross product:

where r is the particle's position vector relative to the fulcrum, and F is the force acting on the particle. The magnitude τ of the torque is given by

where r is the distance from the axis of rotation to the particle, F is the magnitude of the force applied, and θ is the angle between the position and force vectors. Alternatively,

where F⊥ is the amount of force directed perpendicularly to the position of the particle. Any force directed parallel to the particle's position vector does not produce a torque.
It follows from the properties of the cross product that the torque vector is perpendicular to both the position and force vectors. It points along the axis of rotation, and its direction is determined by the right-hand rule.
The unbalanced torque on a body along axis of rotation determines the rate of change of the body's angular momentum,

where L is the angular momentum vector and t is time. If multiple torques are acting on the body, it is instead the net torque which determines the rate of change of the angular momentum:

For rotation about a fixed axis,

where I is the moment of inertia and ω is the angular velocity. It follows that

where α is the angular acceleration of the body, measured in rad·s−2. This equation has the limitation that the torque equation is to be only written about instantaneous axis of rotation or center of mass for any type of motion - either motion is pure translation, pure rotation or mixed motion. I = Moment of inertia about point about which torque is written (either about instantaneous axis of rotation or center of mass only). If body is in translatory equilibrium then the torque equation is same about all points in the plane of motion.
Proof of the equivalence of definitions
The definition of angular momentum for a single particle is:

where "×" indicates the vector cross product, p is the particle's linear momentum, and r is the displacement vector from the origin (the origin is assumed to be a fixed location anywhere in space). The time-derivative of this is:

This result can easily be proven by splitting the vectors into components and applying the product rule. Now using the definition of linearmomentum
p =
mv (if mass is constant) and the definition of velocity


The cross product of any vector with itself is zero, so the second term vanishes. Hence with the definition of force F = ma (Newton's 2nd law),

Then by definition, torque τ = r × F.
If multiple forces are applied, Newton's second law instead reads Fnet = ma, and it follows that
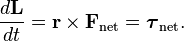
The proof relies on the assumption that mass is constant; this is valid only in non-relativistic systems in which no mass is being ejected.
A particle is located at position r relative to its axis of rotation. When a force F is applied to the particle, only the perpendicular component F⊥ produces a torque. This torqueτ = r × F has magnitude τ = |r| |F⊥| = |r| |F| sinθ and is directed outward from the page.
UNITS
Torque has dimensions of force times distance. Official SI literature suggests using the unit newton metre (N·m) or the unit joule per radian.The unit newton metre is properly denoted N·m or N m. This avoids ambiguity with mN, millinewtons.
The joule, which is the SI unit for energy or work, is dimensionally equivalent to a newton metre, but it is not used for torque. Energy and torque are entirely different concepts, so the practice of using different unit names for them helps avoid mistakes and misunderstandings.The dimensional equivalence of these units, of course, is not simply a coincidence: A torque of 1 N·m applied through a full revolution will require an energy of exactly 2π joules. Mathematically,

where E is the energy, τ is magnitude of the torque, and θ is the angle moved (in radians). This equation motivates the alternate unit namejoules per radian.
In British unit, "pound-force-feet" (lbf x ft), "foot-pounds-force", "inch-pounds-force", "ounce-force-inches" (oz x in) are used, and other non-SI units of torque includes "metre-kilograms-force". For all these units, the word "force" is often left out, for example abbreviating "pound-force-foot" to simply "pound-foot" (in this case, it would be implicit that the "pound" is pound-force and not pound-mass).
Sometimes one may see torque given units that don't dimensionally make sense. For example: g x cm . In these units, g should be understood as the force given by the weight of 1 gram at the surface of the earth. The surface of the earth is understood to have an average acceleration of gravity (approx. 9.80665 m/sec2).